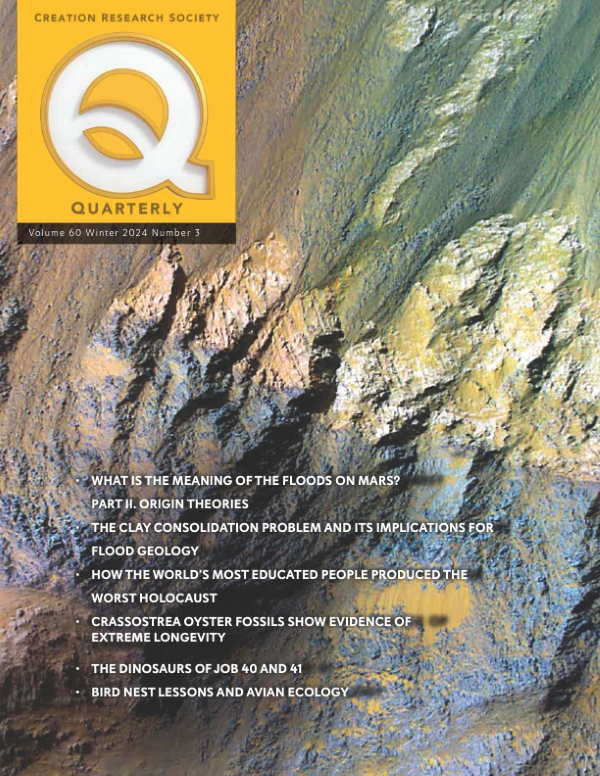
ABSTRACTS
This paper presents evidence that fossil Crassostrea oysters experienced much greater longevity than their modern-day descendants. For extant animals, multiple studies have revealed a positive correlation between longevity and adult body size: the larger the organism's size as an adult, the longer its lifespan. Studies have also demonstrated that organisms that take longer to reach skeletal and/or sexual maturity also tend to have longer lifespans. Fossil Crassostrea oysters are generally larger than their modern-day counterparts, and ontogenetic growth curves suggest longer lifespans and generally longer growth intervals. This evidence should be of great interest to biblical creationists in light of the Bible's claim that humans in the pre-Flood and immediate post-Flood worlds experienced much greater longevities than modern-day humans. Animals may also have once experienced greater past longevity, since whatever genetic or environmental factors were enabling extreme human longevity were likely also operating across the animal kingdom.
Crassostrea Oyster Fossils Show Evidence of Extreme Longevity
Jake Hebert, Frank J. Sherwin, and Richard Overman
Key Words: antediluvian, bivalves, Crassostrea, fossils, giantism, growth bands, longevity, oyster, sclerochronology
Introduction
One of the claims in Scripture that is most ridiculed by skeptics is the Bible's matter-of-fact declaration that, in the pre-Flood world, humans routinely attained ages in excess of 900 years (Genesis 5). Even for some time after the Flood, humans were experiencing lifespans of about 400 years (Genesis 11:12-17), which eventually dropped to 200 years (Genesis 11:18-32), and then gradually decreased to the 70-80 year average lifespan at the time of Moses (Psalm 90:10). Such extraordinary centuries-long lifespans are far beyond our present-day experience. Hence, creationists should be interested in possible corroboration of the Bible's claim in this regard from historical or paleontological data. Previous creationist authors (Patten, 1982; López, 1998) have discussed possible historical and cultural confirmations of these vast ages. Others have discussed possible evidence of greater longevity in post-Flood Neanderthal fossils (Cuozzo, 1998a), as well as a possible connection between greater longevity and past giantism (Patten, 1982; Beasley, 1990; Nelson, 2017).
Crassostrea virginica (Figure 1), also known as the Atlantic or East Coast Oyster, is capable of attaining ages of 20 years with adult sizes of up to 8-10 inches, or 20-25 cm (Wallace, 2022). However, these seem to be exceptional values, with most lifespans and sizes being much less than these (Osborne, 1999; Anonymous a). Other sources (Harzhauser, 2016; Kusnerik et al., 2018) list the maximum lifespan as between 5 and 10 years, and the AnAge Animal Ageing and Longevity Database (de Magalhães, 1997) lists the maximum lifespan for wild Crassostrea virginica oysters as 5 years. They are prized as a seafood, but there is another reason for creationists to love Crassostrea oysters: Crassostrea fossils provide evidence that they were experiencing much greater longevity compared to modern Crassostrea oysters, and they provide an important link suggesting that this was also true for other animal forms.This paper expands on the brief discussion presented by Hebert (2023).
Background: Asymptotic Growth and the von Bertalanffy Growth Equation
Many animals exhibit asymptotic growth; that is, as they mature, their length L asymptotically approaches a maximum value that we designate as L8 (Figure 2). This asymptotic growth can be described mathematically by the von Bertalanffy (1938) growth equation:
Here, t is the time since birth (measured in years) and k is a parameter (with units of years-1) that governs the relative speed with which an organism reaches adulthood. Although k is not a growth rate per se, it is a proxy for growth rate, with high k values representing faster growth and lower k values representing slower growth.
The value t0 is the (theoretical) time at which the organism's length is zero. If the animal has zero size at birth, t0 will be zero. If the creature has a positive, non-zero size at birth, t0 will be a negative number, indicating that the creature had zero size at the beginning of its gestation, -t0years before birth.
Theoretically, Equation describes indeterminate (never-ceasing) growth, since for any finite time t the organism's growth never quite stops. As a practical matter, however, Equation is often used to model both indeterminate and determinate growth, since one can treat the age at skeletal maturity tmature as the time at which the slope of the function becomes arbitrarily small.
Day and Taylor (1997) have criticized use of Equation , arguing that two separate equations are needed to accurately model growth, one for younger ages and a second for older ages. Nevertheless, the von Bertalanffy equation is routinely used by commercial fisheries to estimate age-size relationships for fishes, and it is the growth curve most often used for bivalves (Moss et al., 2021).
Eq. is obtained from a population of organisms. L8 thus represents the average adult body size for the population, obtained from fitting a growth curve to the size-versus-age population data. Since this is a population average, individual specimens in the population will be characterized by L8 values that are both smaller and larger than this.
Given sufficient time, the growth rate of an organism exhibiting determinate growth will become vanishingly small when it reaches skeletal maturity at time t ≈ tmature At this time, the slope of the growth curve approaches zero. Note that tmature is not necessarily the same as the age tsex at sexual maturity, as ages at sexual maturity do not necessarily coincide with ages at skeletal maturity. Indeed, Crassostrea virginica can reach sexual maturity soon after hatching (Anonymous a, Anonymous b), although it continues to grow for much longer than this. Nevertheless, one might reasonably expect higher ages tmature at skeletal maturity to be associated with higher ages of sexual maturity tsex . These points will become important later in our discussion.
Longer Childhood, Longer Life?
Although longevity is influenced by multiple factors, greater longevity in extant creatures has repeatedly been shown to be positively correlated with greater values of tmature. In a study of 124 terrestrial vertebrate taxononomic families, including birds, mammals, reptiles, and amphibians, Ricklefs (2010a) showed that the rate of aging decreased with a number of factors, two of which were increasing gestation period and age at maturity. He also showed that the rate of aging in birds decreased with increasing length of embryonic development. Naturally, one would expect a decreased rate of aging or senescence to imply greater longevity. In order to better ensure that the data met the requirements for a linear least-squares regression, Ricklefs log-transformed his data before performing his correlation, a common practice in such studies. A second study (Ricklefs, 2010b) of 36 bird species and 18 mammal species found that longer embryonic growth rates were positively correlated with decreased rates of aging for both birds and mammals.
A study of 1,456 mammals, birds, amphibians, and reptiles (de Magalhães et al., 2007) showed that age at maturity is positively correlated with maximum adult lifespan. They also showed that postnatal growth rates were inversely correlated with adult lifespan: the faster an organism reached maturity, the shorter its life. Their analysis used a technique called 'phylogenetic independent contrasts' (PIC), which assumes the validity of evolutionary theory. Hence, this may have prejudiced their results by evolutionary assumptions. However, they obtained statistically significant results, both with and without the use of PICs.
Particularly relevant to this study, since we are discussing the molluscan bivalve genus Crassostrea, researchers have repeatedly shown that age at sexual maturity in bivalve mollusks is positively correlated with greater longevity. One particular bivalve, the ocean quahog (Arctica islandica), is the longest-lived molluscan species on Earth (Abele 2008), with a life-span of ~400 years, and even 500 years. Abele et al. (2009) summarize the commonalities previous researchers have observed in extremely long-lived bivalves:
Extremely long-lived bivalves share common and characteristic life history features. The first is an extremely slow and seemingly indeterminate growth in Arctic and Subarctic climates, compared to sympatric species from warmer habitats . . . . A second is the late onset of reproduction which then continues into old age without a post-reproductive phase. [emphases ours]
In today's world, very slow growth and increased lifespans in bivalves are associated with very cold climates and low and seasonal food availability (Palmer et al., 2021). But if slow growth and delayed reproduction were induced for some other reason or reasons, might they still be associated with extreme longevity?
Ridgway et al. (2011) showed a statistically significant positive correlation between the natural logarithm of bivalve adult life span and the natural logarithm of bivalve age at sexual maturity, as well as a statistically significant negative correlation between the natural logarithm of maximum species age and the natural logarithm of the Bertalanffy growth coefficient k. For eight bivalve orders, Moss et al. (2016) also found a statistically significant negative correlation between the logarithm of bivalve lifespan and the logarithm of the von Bertalanffy growth coefficient k.
However, because these studies often involved creatures from different genera, one might perhaps worry that these results only hold when one is comparing lifespans of creatures belonging to different Genesis baramin or 'kinds.' It is well-known that larger, more massive animals (such as elephants) tend to live much longer than smaller, less massive animals (such as shrews). In fact, biologists have long noted that biological timescales, such as lifespan, time to sexual maturity, blood circulation time, etc. are generally proportional to the organism's mass raised to the ¼ power (Lindstedt, 1981; Calder, 1984; Schmidt-Nielsen, 1986). Hence, one would expect more massive species of animals to have longer lifespans and to take longer to reach maturity than less massive species.
But is this merely an inter-species or inter-genera result? Does greater longevity also positively correlate with tmature or with tsex within a single 'Genesis kind'?
Although not as abundant as the evidence cited above, there is some evidence that it does. Most creationists would probably agree that, in general, two species belonging to the same genus are members of the same Genesis kind, even if they have been assigned different species names (Woodmorappe, 1996). A study (Genade et al., 2005) of two fish species of the genus Nothobranchius showed that a Nothobranchius species (N. Kunthae) taking longer to mature lived much longer than the Nothobranchius species (N. furzeri) that matured more quickly (see especially Figure 5 in Genade et al., 2005).
In a study of three-spined stickleback fish (Gasterosteus aculeatus), Lee et al. (2013) found statistically significant positive correlations between pulses of accelerated/slowed growth and decreased/increased longevity. The 2013 study by Lee et al. is particularly noteworthy because it was apparently the first rigorous experimental confirmation of an inverse relationship between growth rate and longevity, as it controlled for the effects of food supply and final adult size:
We used brief (less than 4% of median lifespan) exposure to relatively cold or warm temperatures in early life to deflect juvenile three-spined sticklebacks Gasterosteus aculeatus from their normal growth trajectories; this induced catch-up or slowed-down growth when ambient temperatures were restored, and all groups attained the same average adult size. Catch-up growth led to a reduction in median lifespan of 14.5 per cent [sic], while slowed-down growth extended lifespan by 30.6 per cent [sic]. These lifespan effects were independent of eventual size attained or reproductive investment in adult life.
In this study, the fish all took the same total amount of time to reach maturity, but those experiencing the accelerated catch-up growth had shorter lifespans. It should be noted that both the three-spined stickleback and the freshwater killifish (like Nothobranchius fishes) are often seen as "model systems" for studying vertebrate biology due to a number of factors, including their small body size, short reproduction times, and high fecundity (Reichard and Polacik, 2019; Reid et al., 2021).
Likewise, in a study of six assemblages of extant venerid Phacosoma japonicum bivalves, since reclassified as Dosinia japonica, Sato (1994) showed that greater ages at first and full sexual maturity were consistently associated with greater observed lifespans (Table 1).
Also, Tabatabaie et al. (2011) found that long-lived Ashkenazi Jews were older at first childbirth, suggestive of delayed sexual maturation. However, this last line of evidence is less convincing, due to the many variables that can affect human lifespan.
Delayed Maturation of the Genesis Patriarchs?
In light of these observations, it is striking that the earliest age at which a Genesis 5 patriarch is listed as having a son is 65 (Genesis 5:15, 21). Granted, many of these sons may have not been firstborn, but at least some of them probably were. Given the strength of the human sex drive, it seems very unlikely that the Genesis patriarchs were all becoming sexually mature at 15 or 16 and yet were all deciding to postpone sexual relations for fifty years! It seems far more likely they were becoming sexually mature at much greater ages than do humans today (Patten, 1982; Beasley, 1990). Thus, the greater ages at sexual maturity recorded in Genesis are consistent with expectations from numerous longevity studies: one would expect very long-lived humans to have longer periods of growth than humans with much shorter lifespans. The same is true for the ages of the patriarchs listed in Genesis 11. The earliest age at which a Genesis 11 patriarch is listed as having a son is 29, and most of these listed ages cluster between 30 and 35. Chances are that at least one of these listed sons was a firstborn. By today's standards, 29 or 35 is rather late in life to have a firstborn son.
Whatever conditions in the pre- and immediate post-Flood worlds (lower genetic mutational loads, somewhat higher atmospheric oxygen content, abundant food, etc.) were allowing humans to achieve longer lifespans, those same conditions would likely have also applied to the animal kingdom. Thus we should not be surprised if the fossils themselves provide evidence of much greater animal lifespans than at present.
The Bigger They Are . . . the Longer They Live?
Studies have shown that larger adult body sizes (larger values of L8) are positively correlated with greater longevity (de Magalhães et al., 2007; Wasser and Sherman, 2010; Ricklefs, 2010a; Ridgway et al., 2011). However, there is conflicting evidence in the case of bivalve mollusks. In their study of 56 bivalve species, Ridgeway et al. (2011) found a weak but statistically significant (p = 0.004, 95% level) positive correlation between the natural logarithm of L8 and the natural logarithm of maximum age. However, a larger study by Moss et al. (2016) did not find such a correlation, although Moss et al. did find a positive correlation between tmature and total longevity.
Holm et al. (2016) found that within the family Geometridae, larger moth species tended to live longer than smaller ones. However, it is not clear if this study was free of possible evolutionary assumptions, as this study too made use of phylogenetic independent contrasts (PICs).
The above size-longevity studies compared creatures across 'higher' taxonomic categories, such as classes, orders and families. Thus, the creatures compared often came from different Genesis kinds. Of much greater interest to us are results from within a particular Genesis kind, or baramin. Intra-specific studies by definition are confined to a single baramin, and intra-generic studies are almost certainly confined to a single baramin. However, such intra-specific and intra-generic studies are much less abundant, and results are conflicting. See Marchionni et al (2019) for a review of results from both inter-specific and intra-specific non-creationist longevity studies, including human studies. However, in Sato's (1994) study of six modern Phacosoma japonicum (since reclassified as Dosinia japonica) bivalve assemblages, larger body size was consistently associated with greater ages at sexual maturity and maximum observed ages (Table I), although Sato did not perform tests for statistical significance. Given that greater ages at sexual maturity are often linked with greater longevity and greater size in bivalves, this may be indirect evidence of an intra-species size-longevity connection. Moreover, we shall see in the following sections examples in which (apparently) long-lived fossil oysters are indeed larger than their shorter-lived fossil and modern-day counterparts.
Moreover, there are reasons to suspect a positive correlation between longevity and higher values of L8, even within a baramin. As noted earlier, it has long been observed that biological timescales tend to be proportional to an organism's mass raised to the ¼ power. Consistent with this general result, West et al. (2001) have presented a theoretical justification for expecting an organism's adult body mass M to be proportional to its age at maturity tmature raised to the fourth power:
Of course, higher values of L8 naturally imply higher adult body masses M. Since we have intra-genera experimental justification (Sato, 1994; Genade et al., 2005; and Lee et al., 2013) for expecting delayed maturation (greater ages at maturity) to positively correlate with greater longevity, Eq. implies that we might also expect greater adult body mass M to be positively correlated with greater longevity, as well.
A weakness in this chain of reasoning, however, is that the result of West et al. (2001) does not explore the effect that a change in environmental conditions might have upon longevity within a taxon. In other words, adult body mass within a taxon depends upon age at maturity, but it likely also depends upon factors such as oxygen availability, nutrient availability, etc. These are factors which could conceivably have changed between the pre- and post-Flood worlds. Changes in these variables could conceivably attenuate and/or obscure the general result implied from Eq. .
There is another reason to suspect a positive correlation between greater longevity and larger adult body size (higher values of M and L8). If one considers Figure 2, tmature may be increased by proportionally "scaling up" the size of the organism's growth curve without changing its general shape. Doing so forces tmature to become larger as L8 becomes larger. Since there is some intra-genus evidence (Sato, 1994; Genade et al., 2005; Lee et al., 2013) that tmature is positively correlated with greater longevity, and since one way (but admittedly not the only way) to increase tmature is to increase the organism's adult body size L8, we might also expect greater longevity to be associated with greater adult body size.
Counterexamples
Of course, there are counterexamples to both trends. In a summary of other studies, Marchionni et al. (2019) noted that body masses of mice, horses, dogs, and humans are usually negatively correlated with longevity in intraspecies studies. However, at least in the cases of dogs and horses, some of this could be the result of inbreeding.
Likewise, Miller et al. (2002) found that two out of three wild-derived mice strains were smaller and experienced delayed sexual maturity compared to laboratory strains of mice, yet the wild mice strains were generally longer-lived. They also found that females of these two longer-lived wild strains were also slower to reach sexual maturity. Miller et al. speculated that these results may be due to inbreeding of laboratory strains. So in this particular case, the results were mixed.
Mueller and Mazur (2009) and Samaras (2014) found that greater body size and height were positively correlated with increased human mortality. On the other hand, Brandts and van den Brandt (2019) found a positive correlation between height and longevity for women in the Netherlands but not for men. Given that, as of June 2023, the Dutch people are the tallest in the world (Bostock and Ankel, 2023), this result is intriguing. On the other hand, a very large study (Wormser et al., 2012) found that greater height was associated with a greater risk of cancer but a decreased risk of coronary heart disease and stroke. However, given the multiple factors that can influence human longevity, these results are probably not conclusive, and even the positive results by Tabatabaie et al. (2011) discussed previously should be viewed with some skepticism. Results from experiments under carefully controlled conditions using "model" laboratory animals, like those obtained by Lee et al. (2013) are probably more reliable.
Although it might perhaps seem obvious, it should be noted that body size (specifically, body mass) does not explain all intraspecies and interspecies variation in longevity - it explains much of the variation, but not all of it. For instance, one might naively expect a gorilla (average adult body mass greater than 110 kg) to have a longer lifespan than a human (average adult body mass of 60-80 kg). Yet humans, with their lifespans of around 70-80 years, generally live longer than gorillas (~50 years). So although these trends are real, factors other than mass clearly also influence longevity.
A Working Hypothesis . . . and Predictions
Despite the existence of some contrary data, we assume as a working hypothesis that organism longevity is indeed positively correlated (Figure 3) with both age at maturation tmature and adult body length or height L8. In the absence of a fully-developed theory of longevity, it may not be possible at this time to make an airtight argument for the validity of these assumptions. Nevertheless, they are reasonable. Note also that we are not at this time attempting to explain the cause of these correlations; we are simply accepting them as valid empirical results.
This enables us to make predictions about trends we should see in Crassostrea fossils. We expect fossil Crassostrea oysters to generally show either indirect or direct evidence of greater longevity than modern Crassostrea oysters: larger body sizes and indications of delayed maturation (Figure 3), as well as greater estimated ages at time of death, based on sclerochronological data, discussed in more depth below.
Two of us (Hebert and Sherwin) think evidence for a "high" Cenozoic Flood/post-Flood boundary is overwhelming (Holt, 1996; Oard, 2002a, 2013; Clarey, 2019; and Clarey, Werner and Tomkins, 2022), while the third (Overman) does not have a strong opinion. However, the results of this analysis do not necessarily depend upon the precise location of the Flood/post-Flood boundary. From Scripture, we know that human lifespans decreased dramatically after the Flood and continued to decrease in the following centuries. Hence, we should not be surprised if animal lifespans in general, and Crassostrea lifespans in particular, experienced a similar decline. For instance, suppose some Crassostrea fossils are found within Pliocene rocks. Regardless of whether one dates Pliocene rocks as Flood or post-Flood, if Pliocene rocks date from no more than several hundred years after the Flood, we should expect Pliocene Crassostrea lifespans at a particular location to be greater than those of extant clams, also from that same location, within the same Genesis kind. This is because both pre-Flood and post-Flood human lifespans in the centuries immediately after the Flood were much greater than they are today, and the same would likely be true for animal lifespans.
Complications to Consider
There are complications that should be taken into consideration before doing such a study on bivalves. Bivalve longevity is greatly influenced by factors such as water temperature, with bivalves living in very cold high-latitude waters demonstrating extreme longevity compared to those in warmer, lower-latitude waters. Growth in living Crassostrea specimens is influenced by temperature, salinity, water current velocity, and availability of phytoplanktonic food supply (Kirby and Jackson, 2004). Oysters often colonize together in beds, and crowding is also apparently a factor. According to some online sources, (Osborne, 1999; Wallace, 2022), uncrowded C. virginica oysters can live as long as 20 years. However, most sources list the lifespan for extant C. virginica as much closer to 5-10 years (Harzhauser, 2016; Kusnerik et al., 2018).
It is not possible to completely eliminate the influence of such factors, be we need to minimize their influence as much as possible. Hence, any comparison of modern and fossil bivalve longevity should preferably be restricted to a single location, or to locations very close to one another geographically. At an absolute minimum, the comparison should be restricted to modern and fossil assemblages found within a relatively narrow latitude band. Since we will be comparing late Cenozoic fossil assemblages to modern ones, both creationists and evolutionists would agree that the latitudes of late Cenozoic fossil assemblages would have changed only a little since the time the fossils were formed, regardless of whether one holds to traditional or catastrophic plate tectonics. However, even restricting the analysis to a narrow latitude band does not necessarily eliminate the effects of different water temperatures, for reasons explained later.
Taxonomists have assigned multiple species to Crassostrea, but taxonomists have a well-known tendency to 'oversplit' when classifying organisms, and creationists have long pointed out that creatures within the same genus probably belong to the same Genesis kind (Woodmorappe, 1996), even if they have been classified as different species. Hence, comparisons of different Crassostrea species within the genus are still likely to represent valid comparisons within a single Genesis kind. In fact, as we discuss later, even some evolutionists have suggested that modern Crassostrea oysters are direct descendants of much larger (and apparently much longer-lived) fossil Crassostrea forms.
This paper presents evidence that Crassostrea longevity was once much greater in the past. However, in order to understand the results, a brief introduction to the field of sclerochronology is in order.
Sclerochronology: An Introduction
Sclerochronology is the study of features such as chemical and periodic structures in the skeletal portions of animals that grow by accretion (layering), such as bivalves (Killam 2018, p. vi):
Bivalve shells contain growth lines which are formed as a result of periodic environmental or physiological stress, analogous to tree rings. The study of these regular growth increments in the hard parts of bivalves and other calcifying organisms is called Sclerochronology
The field has been widened to include the study of periodic bands in numerous aquatic and terrestrial taxa, including coralline algae, fish and gastropods (Jones et al. 1989).
Evolutionary scientists think (Trofimova et al. 2020, p. 2) "the full potential of sclerochronology has yet to be realized." Moss et al. (2021, p. 1) stated:
Not only can sclerochronological data help to address long-standing questions in paleobiology, but they can also bring to light new questions that would otherwise have been impossible to address. For example, growth rate and life-span data, the very data afforded by chronological growth increments, are essential to answer questions related not only to heterochrony and hence evolutionary mechanisms, but also to body size and organism energetics across the Phanerozoic.
We agree with evolutionary scientists that sclerochronology has untapped potential, but for different reasons. We think it can assist in determining the effects of the Flood thousands of years ago and up to recent times. We see this field as helping to determine faunal periodic structures - and lifespans - in the pre-Flood and post-Flood worlds. As noted in the above statement by Killam, sclerochronology is much like dendrochronology, and, like dendrochronology, caution should be employed when evaluating data.
Wide bands in mollusk bivalve shells are produced during times of rapid growth, and narrow bands are produced during times of reduced growth. These times of growth reduction or cessation are thought to coincide with physiological stress (Buick and Ivany, 2004). These bands may occur at different times of the years for the same mollusks in different settings (Moss et al., 2021; Jones and Quitmyer, 1996).
It has long been known (Jones 1981) that not all bands in a mollusk shell are annual. Moss et al. (2021) note that periodic lines in shells have been shown to be tidal, daily, fortnightly, monthly, and annual. Some bands, called disturbance lines, can result from storms or attack by predators (Moss et al., 2017).
External bands on the outside of a bivalve shell (or valve) are often disturbance bands, and counting them can lead to erroneous age estimates (Moss et al., 2017). Internal growth bands are thought to be more accurate proxies for age, and these are observed by cutting open the valve in cross-section (Moss et al., 2017).
Can We Trust the Band Counts?
This raises an extremely important question: can bivalve annual band counts be trusted? Creationists are generally distrustful of claims that dendrochronology can provide accurate timelines going back thousands of years (Woodmorappe, 2003; Hebert, Snelling, and Clarey, 2016; Woodmorappe, 2018). If creationists accept these oyster band counts as being reasonably accurate, are we being inconsistent?
Due to uncertainties about the past, it would be a mistake to put unqualified trust in this or any other such counting method. However, for the following reasons, we can be reasonably confident that these band counts are legitimate, albeit imperfect, proxies for age.
Due to its importance to the seafood industry, modern Crassostrea virginica lifespans are likely well-established by direct observation. This gives us additional confidence that, in the analysis below, the ages inferred from sclerochronological analysis of modern Crassostrea virginica specimens are reasonably accurate. And in the case of Crassostrea oysters, we are not attempting to count thousands, or even hundreds, of presumed annual bands, as is the case for ice core and tree ring dating. Rather, we are attempting to count, at most, a few dozen such bands. Moreover, our analysis does not depend upon the problematic process of 'cross-matching' banding patterns in one oyster assemblage to those in another (Woodmorappe, 2003, 2009, 2018).
Also, disturbance bands are not likely to be mistaken for periodic bands, due to their random nature (Moss et al., 2017). Moreover, in the case of Crassostrea oysters, Zimmt et al. (2019) identified subtleties in band appearance that can be used to distinguish annual bands from non-annual bands. Moss et al. (2021) noted that annual growth bands are found in almost all extant bivalves outside the tropics, forming in response to seasonal extremes in variables such as temperature, salinity, and food availability. Moss et al. (2017, p. 367) state that annual bands are "unambiguous" if formed in a highly-seasonal environment.
Geochemical Variations Can Aid In Counting
Moreover, geochemical variations may help identify seasonal patterns, even when those seasonal variations are not as pronounced, as they can serve as a "check" on annual band counts (Moss et al., 2021, p. 3):
Without independent temporal calibration, the assumption that growth bands are annual is not always a safe one - in some cases, visible growth increments bear no clear relation to intra-annual environmental variation. Geochemical variation along the axis of growth, however, often provides a means by which to confirm the timing of visible growth bands and reveal the annualcycle , thereby allowing calculation of growth rates and/or determination of ontogenetic age. [emphasis ours]
Geochemical variation refers to changes in a quantity like the oxygen isotope and carbon isotope ratios (d18O and d13C, respectively). Although creationists have been skeptical of the level of climate detail that mainstream paleoceanographers claim to be able to infer from ancient oxygen isotope (d18O) values (see Oard 1984, Vardiman 2001, Oard 2003, and Hebert 2021 for a discussion), we acknowledge that d18O values are rough proxies for temperature, provided that the shell from which the d18O values are obtained was formed in isotopic equilibrium. This assumption would likely break down during the Flood itself, potentially invalidating the interpretation of the very last band or bands that might have formed during the Flood. However, this assumption would likely have been valid in the pre-Flood and post-Flood worlds. Therefore, if seasonal variation was present in the pre-Flood world, even if less pronounced than today, variations in d18O values within bivalve shells could help identify annual bands within those pre-Flood bivalves. The same would likely be true for the post-Flood world.
Moreover, the use of d18O variations as a "check" on annual counts within a shell does not require a precise climatic interpretation of those variations. Since only observation of repeating maximum and minimum d18O or d13C values is required for this purpose, this avoids the potential perils of attempting to deduce precise climate information (Moss et al., 2021), which requires unprovable assumptions about the past (Oard, 1984; Hebert, 2021).
Indeed, Kirby (2000) demonstrated that annual Crassostrea bands can likely still be resolved, even when seasonal isotopic variation is less pronounced:
d18O and d13C profiles across skeletal growth increments in two well-preserved [North Carolina Late Oligocene] C. gigantissima shells show significant differences with profiles from Pleistocene and Recent C. virginica [from Chesapeake Bay and the Mississippi Delta]. Significantly higher d18O and d13C values and smaller seasonal isotopic ranges with less variability show that C. gigantissima lived in a more fully marine environment than C. virginica.
In passing, it should be noted that this is not the only possible interpretation of the data. It may be that C. gigantissima simply lived in an environment with less-pronounced seasonal differences than C. virginica, an intriguing possibility in light of creationist suggestions that seasonal extremes were less-pronounced in the pre-Flood world (Whitcomb and Morris, 1991), and that even the post-Flood Ice Age climate was relatively equable (Oard 1990, 2005). Kirby found that over eight apparent growth cycles, d18O and d13C minima coincided, but the maxima were slightly offset.
Other studies have demonstrated the usefulness of geochemistry in identifying annual bands. Buick and Ivany (2004) found that for Cucullaea raea bivalves from Antarctica, troughs in d18O values consistently coincided with narrow bands corresponding to times of growth reduction or cessation, and this pattern held for 17 such growth cycles. These growth cessation bands were visible to the naked eye, with widths on the order of 0.25 mm thickness. The intervening bands corresponding to times of growth were usually about a millimeter wide. The reverse pattern held for d13C variations, with peak d13C values coinciding with the narrow band times of growth reduction. Ivany and Runnegar (2010) showed that oxygen isotope values varied seasonally over six years of growth within an early Permian bivalve. Zimmt et al. (2019) showed a strong correlation between d18O and seasonal variations in Pleistocene Crassostrea virginicaoysters from the mid-Atlantic United States. In a study of Recent Crassostrea oysters from Altamaha Sound, Georgia, Andrus and Crowe (2000) showed that dark zones (representing times of faster growth during cooler months) consistently had higher d18O and d13C values. They concluded that banding patterns were generally seasonal, but dark bands could form at any time due to extreme heat or floods.
Band Counts Likely Underestimate True Age
Both creation and evolutionary scientists would agree that band counts likely underestimate true bivalve age for a number of reasons. First, biological ages are often assigned to Crassostrea oysters by counting periodic increments in the ligament area. In both fossil and modern oysters, the earliest part of the ligament area, representing the first two years of growth, is often missing (Kirby, 2001), but because this is true in both the fossil and modern specimens, this will probably not affect the comparison much.
Second, in very-long lived bivalves, bands become exponentially thinner over time, making them harder to see, which means the true age can be underestimated. Moss et al. (2017, p. 373) give an example in which the last 5 millimeters of growth in one species represented 20 out of 39 years of its lifespan.
In the following sections, we will be comparing data from Miocene, Pliocene, and Eocene Crassostreaoysters to data from Pleistocene and Modern Crassostrea oysters. If the Flood/post-Flood boundary is 'high' in the Cenozoic, then the Miocene, Pliocene, Eocene, and perhaps some of the Mid- or Upper Pleistocene (Holt, 1996) fossil oysters were killed during the Genesis Flood. This means they did not die natural deaths. In this case, sclerochronological band-counting can only approximate their ages at death, but this does not necessarily tell us anything about their true lifespan potential, at least not directly. Hence, these age estimates are likely underestimating true lifespan potential, perhaps significantly.
Even if one attributes upper Cenozoic fossilization to post-Flood catastrophic flooding, the catastrophic nature of that record is still consistent with this conclusion. Some fossil bivalves have their valves clamped shut, a strong indication that they were still alive when they were buried, consistent with catastrophic death and burial (Good, 1998; Hoesch and Austin, 2004). Hence, inferences from fossil Crassostrea data will likely underestimate true lifespan potential, regardless of whether those fossils are attributed to Flood or post-Flood catastrophism. Also, unless one wishes to assume that oysters were dying promptly upon reaching maturity, there is no good reason to assume that the ages at maturity necessarily represent the total potential lifespan. It seems more likely that the total potential lifespan would be greater.
Error Probably Insufficient to Affect Analysis
Clearly, there will be some error in bivalve band counts. Neither creationists nor evolutionists expect these counts to be perfect. But any such error will only invalidate the results if disturbance bands are both very numerous and very hard to identify. For the reasons given above, we do not think this will be the case.
Also, there does not seem to be any reason evolutionary paleontologists would want to favor greater oyster band counts over smaller ones, or vice versa . Thus, the problem of bias, due to pre-conceived expectations, that affects ice core and seafloor sediment age assignments (Oard, 2005; Hebert, 2021), is minimized.
Some paleontologists seem very confident that band counts can be reliable age indicators for bivalves in general and for Crassostrea oysters in particular (Kirby, 2001; Moss et al., 2021), regardless of location, provided that the analysis is done with appropriate caution. Zimmt et al. (2019) are more cautious, arguing that this has only been demonstrated to be true for Pleistocene Crassostrea oysters from the mid-Atlantic plain (USA). Nevertheless, there seems to be general agreement among sclerochronologists that, despite possible uncertainties, these banding counts are sufficiently accurate to conclude that mid-Atlantic Pleistocene Crassostrea oysters were indeed longer-lived than their modern-day counterparts living in the same area (Zimmt et al., 2019; see also the studies by Norton, 2021, and Falb, 2022).
Finally, evidence that Crassostrea oysters experienced much greater longevity than extant Crassostrea oysters does not depend entirely upon the band counts. We have already listed some reasons to suspect that larger adult body sizes are also indicators of great longevity, and we present examples below in which the fossil Crassostrea forms are muchlarger than the modern forms.
Fossil vs. Modern Crassostrea: Virginia, Maryland, and North Carolina (USA)
Kusnerik et al. (2018) performed a study using more than a thousand Pleistocene Crassostrea oyster fossils obtained from five assemblages in southern Maryland, Virginia, and North Carolina (Chesapeake Bay region, USA). This geographical area spanned ~2.7° in latitude. These specimens were supplemented by specimens from the Virginia Museum of Natural History. These were then compared with both modern specimens and specimens from the American colonial period. They excluded specimens with shell heights less than 35 mm, in order to avoid inclusion of immature oysters (spat) in the study, and they took care to ensure that all oysters came from environments with similar salinities (15-30 ppt).
Because the shell data were, in most cases, non-normally distributed, they used the Mann-Whitney U test to check for differences in shell height across Maryland, Virginia, and North Carolina. They found statistically significant size differences, shown in Table 1I. They also found statistically significant differences in growth rates between the modern, colonial, and Pleistocene specimens, with the colonial and modern oysters growing considerably faster than the Pleistocene specimens.
Of particular interest to this study, they found that Maryland Late Pleistocene (LP) oysters were significantly larger than both colonial and modern oysters (Figure 4). Virginia Middle Pleistocene (MP) oysters were also significantly larger than both colonial and modern oysters (Figure 5). North Carolina MP oysters were significantly larger than LP oysters, but not modern ones (Figure 6). The difference between LP and Modern North Carolina specimens was also statistically significant.
The Pleistocene oysters were also longer lived than the Modern and Colonial oysters. Age estimates were obtained by counting gray bands in the cross-sections of bisected hinges. In Maryland, Pleistocene oysters attained to ages of 12 years, whereas the modern and colonial oysters rarely lived beyond 5 years. In Virginia, Pleistocene oysters lived beyond 20 years, with colonial and modern oysters rarely living beyond 5 years. For the North Carolina specimens, Pleistocene oysters did not live beyond 4 years of age, and moderns did not attain to 3 years of age (there were no colonial North Carolina specimens).
Generally, Pleistocene Crassostrea oysters were larger, took longer to mature, and were longer-lived than more recent oysters. This supports our working hypothesis that, within a biblical kind (and not merely across kinds), larger body size does indeed seem to correlate with greater longevity. Admittedly, some of this decrease in longevity and body size could have been an adaptive response to cooling oceans as the Ice Age came to an end. However, for the Maryland and Virginia oysters, the decrease in longevity and body size continued from the American Colonial Age to Modern times, long after the post-Flood Ice Age came to an end.
The results in Figure 6 partially contradict this expectation, however, as one would expect longer-lived Late Pleistocene (LP) oysters to be larger than shorter-lived Modern oysters. However, it should be noted that Pleistocene and Modern longevities in North Carolina were much more similar (no greater than 4 and 3 years, respectively) than they were in Virginia and Maryland. Hence, one might expect their body sizes to be more similar, as well.
In the next example we see additional evidence that Pleistocene Crassostrea oysters were longer lived than recent specimens, and that fossils pre-dating the Pleistocene were both bigger and longer-lived than both Pleistocene and recent specimens.
Fossil vs. ModernCrassostrea: North Carolina and Virginia (USA)
Kirby (2001) counted internal growth ligaments to construct growth curves for Tertiary (Upper
Miocene) and Quaternary (Pleistocene and 'Recent') Crassostrea specimens. The Miocene species, named
Crassostrea titan because of its large size, was collected from two locations in California. The Pleistocene and
'Recent' specimens, Crassostrea virginica (Figure 1) were obtained from Virginia and North Carolina,
respectively. All specimens were obtained from between 34.6 and 36.8° north latitude.
Although Kirby does not specify whether or not the Pleistocene strata are Early-, Mid-, or Late- Pleistocene, he does refer back to Spencer and Cambell (1987), who state that the Virginia clams are from Late Pleistocene marine sediments. The Late Miocene specimens were dated (Kirby, 2001) as between 8 and 12 million years old on the uniformitarian timescale.
In agreement with expectations, the Recent and Late Pleistocene oysters were generally much smaller and (apparently) shorter-lived than the Miocene specimens (Figures 7, 8, and 9). It is also evident from Figure 8 that the slopes of the Miocene growth curves approach zero at much greater ages than do the Recent and Late Pleistocene specimens, indicating that the Miocene oysters took much longer to mature. Kirby performed a Mann-Whitney U test that showed a statistically significant difference (p < 0.05) in lifespan between the Miocene C. titan oysters and the more recent (Modern and Late Pleistocene) C. virginica oysters. The results generally agree with our expectations. However, the growth curve for the Recent specimens indicated a larger average adult body size than that possessed by the Late Pleistocene specimens, even though the Pleistocene specimens were longer-lived. This is contrary to expectations, if one assumes that larger body sizes are generally associated with greater longevity.
It should be noted that the average age of the Recent specimens was 3 years, the average age of the Miocene-1 specimen was ~7 years, and the average age of the Miocene-2 assemblage was 8 years (Figure 8). Unless Kirby over-counted the true number of annual bands in the Miocene assemblages by a factor of more than 2, it is difficult to avoid the conclusion that the Miocene Crassostrea specimens were indeed longer lived than the Recent specimens.
In today's world, at a given latitude, California Pacific coastal waters are considerably cooler than waters off the North American east coast (Herbert et al., 2016). For instance, according to the website of San Diego's Fleet Science Center (fleetscience.org, accessed October 31, 2023), the waters off the coast of San Diego are about 8.9° Celsius colder than waters off the coast of Charleston, South Carolina. This is because coastal California waters originates near Alaska, while waters off the eastern coast originate from the warmer Caribbean. Can uniformitarians attribute the extreme longevity of these California Miocene specimens to much colder Pacific water temperatures? Probably not. Although uniformitarians believe a cooling trend began in the Miocene about 7 million years ago, they think the Miocene 12 to 8 million years ago was considerably warmer than today's world (Holbourn et al., 2018). A recent uniformitarian temperature reconstruction (Herbert et al. 2016, especially their Figure 2f) concluded that, on average, sea surface temperatures at the southern California margin were between 7 and 9° Celsius warmer than in today's world. Hence, by uniformitarian reckoning, California coastal sea surface temperatures at this time would have been comparable to Atlantic sea surface temperatures today. Moreover, Kirby did not even suggest colder waters as a possible explanation, as he assumed that Late Miocene waters would be relatively warm. Nor can these great longevities be due to colder temperatures within a creationist framework, as most creationists think that the pre-Flood and immediate post-Flood worlds were characterized by relatively warm oceans.
Additional Crassostrea Observations
Some comments are in order before proceeding to the next example. Evolutionary scientists have acknowledged that the very large sizes of Tertiary Crassostrea oysters could be due to greater longevity (Kirby 2001, p. 84):
The reasons for producing such large shells have not been well understood, but previous studies have considered them to be a consequence of longer life spans due to the absence of human predation (Stenzel 1971), the presence of photosymbionts (Jones et al. 1988; but see Cowen 1983), or life on muddy substrates (Chinzei 1995). [emphasis ours]
Moreover, not only are the valve heights of the Miocene Crassostrea greater, but so are their valve (shell) thicknesses when compared to Recent and late Pleistocene specimens (Kirby's Figure 3A, not shown). Kirby suggests these large thicknesses were a defense mechanism against predation, but this is not the only possible explanation.
It should be noted that, in this and the following examples, some of these oysters were growing very rapidly in absolute terms, faster than many modern-day extant oysters. That is, the length or mass they gained per year was higher than that experienced by comparable extant organisms at the same ontogenetic stage. Kirby summarizes (2001, p. 89):
Estimation of life spans from ligamental increments and measurements of valve thicknesses and height shown that the C. titan specimens lived two to three times longer and grew significantly faster than the C. virginica specimens.
Even though they were growing rapidly in absolute terms (millimeters or grams of growth per year), attainment of their larger adult body sizes required longer growth intervals than extant forms. Hence, the net effect was longer growth intervals, despite these higher absolute growth rates.
In a study of Pliocene Crassostrea oysters from the tropical Americas, Kirby and Jackson (2004) concluded that Miocene-Pliocene C. cahobasensis growth rates (in mass per year) were 2.5 times faster than those of Quaternary C. virginica and C. columbiensis. They concluded that the fast-growing C. cahobasensis went extinct, while the more slowly-growing C. virginica and C. columbiensis survived. But what if C. virginica and C . columbiensis are direct and more slowly-growing descendants of C. cahobasensis? We return to this particular example later below.
Eocene Crassostrea oysters from Texas (Finch, 1824) and Georgia (Edwards, 2016) can also be quite large and have been given the species names Crassostrea gigantissima (Figure 10). The extinct Micoene-Pliocene C. ingens from New Zealand was also classified as a giant Crassostrea oyster. According to Wikipedia, it had shell heights of 200 to more than 300 mm, comparable to the heights of the Miocene Crassostrea whose growth curves are shown in Figure 8. Unfortunately, it was not possible to confirm this size for C. ingens from a more scholarly source. It should also be noted that C. ingens was recently reclassified, although we have been unable to find its new taxonomic designation. Likewise, C. gigas was recently reclassified as Magallana gigas (World Register of Marine Species).
A study of 1,121 complete shells in a Miocene C. gryphoides bed in Austria (48° N latitude) showed that C. gryphoides apparently lived for at least 40 years (Harzhauser et al., 2016) and attained heights of about 80 cm (Figure 11). Before it's reclassification as M. gryphoides , it was the largest known Crassostrea oyster, fossil or modern. Given that its adult size is so much larger than the Miocene specimens shown in Figures 8 and 11, it probably does belong to a different genus than Crassostrea.
Interestingly, the vast majority of the clams in this Austrian assemblage lived less than ten years and grew to be 'only' about 30 cm in length, an intriguing observation in light of Robert Carter's (2023) recent suggestion that extremely long-lived individuals should be very rare in a given population.
Because of its recent change in classification, and because these Austrian C. gryphoides (or M. gryphoides) oysters were found at significantly higher latitudes than those in Kirby's study, it is probably inappropriate to directly compare the C. gryphoides growth curve to the other growth curves in Figure 11, even though they have all been depicted on the same graph.
However, Crassostrea gigas, the Pacific giant oyster, has been reclassified as Magallana gigas. The paleoenvironment for the Austrian M. gryphoides shell bed has been described (Harzhauser et al., 2016, p. 1225) as "comparable to the settings of modern Crassostrea reefs in the subtropical parts of the Asian Pacific." In that light, it is worth noting (Anonymous b, California Sea Grant, no date) that the Pacific giant oyster ( C. gigas or M. gigas) has been known to grow to a size of 38 cm (15 inches). However, Cowles (2005) states M. gigas has a maximum size of 25 cm and "may live 20 years or more." According to Nehring (2011), unharvested C. (or M.) gigas oysters can live to be 30 years old. Yet Figure 11 shows that M. gryphoides took 40 years just to mature. So despite apparently living in a comparable subtropical environment, the Austrian fossil M. gryphoides was approximately twice as large and longer-lived than the extant giant Pacific oyster M. gigas!
It should also be noted that all the modern Crassostrea growth curves from France, Bangladesh, Mexico, and the Atlantic coast of the United States (curves 2-5 in Figure 11) indicate smaller body sizes and accelerated maturation compared to the two Miocene Crassostrea curves from California (curves 1), despite their wide latitude distribution and the taxonomic uncertainty of the C. gigas or M. gigas specimen from France (curve 5).
Some evolutionists have acknowledged a close link between fossil and extant Crassostrea clams, despite their assignment to different species. Sohl and Kauffman (1964, p. H1) argued that C. virginica may be the modern representation of both the Cretaceous C. cusseta (now a defunct classification) and the Eocene C. gigantissima:
C. cusseta is the terminal Cretaceous member of the C. soleniscus lineage in [American] gulf coast sediments; the lineage continues, however, with little basic modification, through the Cenozoic, being represented in the Eocene by G. gigantissima (French) and probably, in modern times, by C. virginica (Gmelin).
Lawrence (1991, p. 342) goes even further, saying, C. gigantissima "is most certainly the direct ancestor of C. virginica." If C. gigantissima is the "direct ancestor" of today's C. virginica, isn't this just another way of saying that C. virginica and C. gigantissima are really the same Genesis 'kind' or baramin? The much greater sizes and growth intervals of C. gigantissima compared to C. virginica are indirect evidence that C. gigantissima was experiencing much greater longevity than its modern-day descendants.According to the World Registry of Marine Species (marinespecies.org), C. virginia commonly has a length of about 8.5 cm, whereas C. gigantissima was much, much larger. Some C. gigantissima specimens (Figure 10) were more than 55 cm in length (Edwards, 2016, p. 5).
In passing, we should note that if we accept the respective taxonomic reclassifications of C. gigas and C. gryphoides to M. gigas and M. gryphoides, we now have evidence of extreme past longevity from twodifferent oyster genera, rather than just one.
Crassostrea Oysters from the Tropical Americas
Kirby and Jackson (2004) compared body sizes and growth rates of 542 Miocene, Pliocene, and Quaternary juvenile (ages less than 7 years) Crassostrea oysters from the tropical Americas. Their study included Recent, Holocene, and Pleistocene C. virginicafrom Venezuela, Trinidad, Panama, Barbados, and Costa Rica; Recent and Pleistocene C. columbiensis oysters from Panama; Pliocene and Miocene C. cahobasensis from Venezuela and Panama, and Pliocene Crassostrea aff. C. virginica specimens, whose taxonomic status was uncertain, from Trinidad, Jamaica, and Venezuela (Table III). With the exception of the Jamaica specimens (18° N latitude), all oyster specimens were collected from a latitude band of ~4.6° (Figure 1 in Kirby and Jackson, 2004).
Kirby and Jackson (2004) calculated estimated carbonate content of these shells. They also used an allometric relationship to estimate total biomass for the specimens. The Miocene and Pliocene C. cahobasensis specimens were consistently larger, at all ages, than the Quaternary C. virginica and C. columbiensis oysters (Figure 12). This was true regardless of whether estimated carbonate content or biomass was used as a measurement of body size. With the exception of some Recent C. columbiensis oysters from Panama, most of the Recent oysters seem to have been C. virginica . It is thus striking that the Recent C. virginica oysters are consistently smaller than the Pleistocene C. columbiensis oysters, which are consistently smaller than the Miocene and Pliocene C. cahobasensis oysters. With the one exception of the Pliocene C. aff. C. virginica oysters, whose taxonomic status is uncertain, the pattern is consistent with our working hypotheses that adult body sizes are associated with longer-lived organisms, and that both human and animal longevities were decreasing after the Flood.
Note also that a Pacific-Atlantic temperature gradient is insufficient to explain these size differences, as inspection of the data in Table 1 and Figure 1 in Kirby and Jackson (2004) show that nearly all the Crassostrea specimens were collected from locations on the Atlantic side of Central and South America.
Concluding Remarks
Hebert (2023) accumulated preliminary evidence of extreme longevity in fossil forms (bivalves, alligators and crocodiles, sharks, and possibly birds). Since humans once experienced much greater longevity, and since whatever conditions enabled this longevity likely also affected the animal kingdom, this result makes good sense in light of the Bible's testimony. It also has the potential to be a great encouragement to Bible-believing Christians, especially if it can be shown that this longevity was experienced by a wide array of organisms. Although these preliminary results are potentially encouraging, they require more in-depth analysis, and potential objections to these arguments need to be addressed. This paper was an attempt to do that in the case of Crassostrea oysters, specifically. This paper also helps illustrate some of the steps suggested by Overman (2021) in performing creationist scientific research. Specifically, the Bible's statements regarding past human longevity were taken seriously and used to derive conclusions that could be (and were) tested.
Because bivalve fossils are extremely abundant (Morris and Sherwin, 2011), creation researchers could potentially do original field work in this area. However, the already-published research in the mainstream paleontological literature should not be neglected, as it is likely that much evidence of such longevity has already been published but has simply been overlooked by evolutionary researchers.
Finally, it is worthwhile to here honor the contributions of earlier generations of creationists. Many creationists, including Henry M. Morris (1993), noted the very large sizes of fossil creatures compared to their living counterparts. Donald W. Patten (1982) and Greg Beasley (1990) both suggested a link between larger body sizes, delayed maturation, and greater longevity. The deduction of the positive correlation between longevity and delayed maturation is particularly impressive, because any scientific evidence for such a link that may have existed in the early 1980s and 1990s was probably not well known. Thus Patten and Beasley seem to have deduced that delayed maturation would be linked to greater longevity solely from the Scriptural data in Genesis 5 and 11! Beasley in turn acknowledged the work of Donald Patten and the influence on his thinking of articles by creation pioneers like Gary Parker, Duane Gish, Joseph Dillow, and Erich von Fange. Jack Cuozzo (1998b) also suggested that ancient humans matured more slowly than modern humans. Although not all their ideas were necessarily correct, these creationists were, I believe, "on the right track." It has taken a long time, but data from sclerochronology are now making it possible to finally test a creationist prediction made more than forty years ago. Although more analysis is needed before drawing firm conclusions, the data presented here suggest that this predicted linkage of greater longevity, larger body size, and delayed maturation may indeed have been correct.
References
Abele, D., J. Strahl, T. Brey, and E. Philipp. 2008. Imperceptible senescence: Ageing in the ocean quahog Arctica islandica. Free Radical Research 42(5): 474-480.
Abele, D., T. Brey, and E. Philipp. 2009. Bivalve models of aging and the determination of molluscan lifespans. Experimental Gerontology 44(5): 307-315.
Andrus, C.F.T., and D.E. Crowe. 2000. Geochemical analysis of Crassostrea virginica as a method to determine season of capture. Journal of Archaeological Science 27(1): 33-42.
Anonymous a. Eastern Oyster (Crassostrea virginica). Texas Parks and Wildlife . https://tpwd.texas.gov/huntwild/wild/species/easternoyster/ (accessed August 30, 2023).
Anonymous b. Sea Grant California. Seafood Profiles: Pacific Oyster. University of California San Diego. https://caseagrant.ucsd.edu/seafood-profiles/pacific-oyster (accessed August 11, 2023).
Beasley, G. 1990. Pre-flood giantism: A key to the interpretation of fossil hominids and hominoids. Journal of Creation 4(1): 5-55.
Bostock, B., and S. Ankel. Ranked: The countries with the tallest people in the world. Business Insider. https://www.businessinsider.com/tallest-people-world-countries-ranked-2019-6 (posted June 7, 2023, at businessinsider.com; accessed December 18, 2023).
Brandts, L., and P.A. van den Brandt. 2019. Body size, non-occupational physical activity and the chance of reaching longevity in men and women: findings from the Netherlands Cohort Study. Journal of Epidemiology and Community Health 73(3): 239-249.
Buick, D.P., and L.C. Ivany. 2004. 100 years in the dark: Extreme longevity of Eocene bivalves from Antarctica. Geology 32(10): 921-924.
Calder, W. A. III. 1984. Size, Function, and Life History. Harvard University Press, Cambridge, MA.
Carter, R. 2023. The extreme rarity of long-lived people in the post-Flood era. Journal of Creation 37(2): 63-67.
Clarey, T.L. 2019. South Caspian Basin supports a Late Cenozoic Flood boundary. Journal of Creation 33(3): 8-11.
Clarey, T.L., D.J. Werner, and J.P. Tomkins. 2022. Globally extensive Cenozoic coals indicate high post-Flood boundary. Journal of Creation 36(1): 13-15.
Cowles, D. 2005. Magallana gigas (Thunberg, 1793). University of Walla Walla. https://inverts.wallawalla.edu/Mollusca/Bivalvia/Ostreoida/Ostreidae/Crassostrea_gigas.html (posted at https://inverts.wallawalla.ed ; accessed October 16, 2023).
Cuozzo, J. 1998a. What happens to the craniofacial structures of humans who live past 100 years? Neanderthal Similarities. In R.E. Walsh (editor). Proceedings of the Fourth International Conference on Creationism, pp. 103-119. Pittsburgh, Pennsylvania: Creation Science Fellowship, Pittsburgh, PA.
Cuozzo, J. 1998b. Buried Alive: The Startling Truth About Neanderthal Man . Master Books, Green Forest, AR.
Day, T., and P.D. Taylor. 1997. von Bertalanffy's growth equation should not be used to model age and size at maturity. The American Naturalist 149(2): 381-393.
de Magalhães, J.P. 1997. AnAge: The Animal Ageing and Longevity Database. Human Ageing Genomics Resources. University of Liverpool. https://genomics.senscence.info/species/index.html.
de Magalhães, J.P., J. Costa, and G.M. Church. 2007. An analysis of the relationship between metabolism, developmental schedules, and longevity using phylogenetic independent contrasts. The Journals of Gerontology: Series A 66(2): 149-160.
Edwards, E.O. 2016. Shell Bluff - A fossiliferous ridge, the site of the extinct oyster Crassostrea gigantissimaand history of its identification. Georgia Journal of Science 74(2), Article 12: 1-14.
Falb, O. 2022. Growth rates and sclerochronology of modern Crassostrea virginica from the lower Chesapeake Bay (southeastern Virginia). Geology Senior Theses . William & Mary. Paper 42.
Finch, J. 1824. Geological essay on the Tertiary formations in America. The American Journal of Science and Arts 7: 31-42.
Genade, T., M . Benedetti, E. Terzibasi, P. Roncaglia, D. R. Valenzano, A. Cattaneo, and A. Cellerino. 2005. Annual fishes of the genus Nothobranchius as a model system for aging research. Aging Cell 4(5): 223-233.
Good, S.C. 2004. Paleoenvironmental and paleoclimatic significance of freshwater bivalves in the Upper Jurassic Morrison Formation, Western Interior, USA. Sedimentary Geology 167(3): 163-176.
Harzhauser, M., W.E. Piller, S. Müllegger, and O. Mandic. 2016. Age structure, carbonate production and shell loss rate in an Early Miocene reef of the giant oyster Crassostrea gryphoides. Biogeosciences 13: 1223-1235.
Hebert, J., A.A. Snelling, and T.L. Clarey. 2016. Do varves, tree-rings, and radiocarbon measurements prove an old Earth? Answers Research Journal 9: 339-361.
Hebert, J. 2021. The Ice Age and Climate Change: A Creation Perspective . Institute for Creation Research, Dallas, TX.
Hebert, L. III 2023. Allometric and metabolic scaling: Arguments for design . . . and clues to explaining pre-Flood longevity? Proceedings of the Ninth International Conference on Creationism : Volume 9, Article 18. Whitmore, J. (editor). Cedarville University, Cedarville, OH.
Herbert, T.D., K.T. Lawrence, A. Tzanova, L.C. Peterson, R. Caballero-Gill, and C.S. Kelly. 2016. Late Miocene global cooling and the rise of modern ecosystems. Nature Geoscience 9: 843-847.
Hoesch, W.A., and S.A. Austin. 2004. Dinosaur National Monument: Jurassic Park or Jurassic Jumble? Acts & Facts 33(4).
Holbourn, A.E., W. Kuhnt, S.C. Clemens, K. G.D. Kochhann, J. Jöhnck, J. Lübbers, and N. Andersen. 2018. Late Miocene climate cooling and intensification of southeast Asian winter monsoon. Nature Communications 9(1584): 1-13.
Holm, S., R.B. Davis, J. Javoiš, E. Õunap, A. Kaasik, F. Molleman, and T. Tammaru. 2016. A comparative perspective on longevity: the effect of body size dominates over ecology in moths. Journal of Evolutionary Biology 29(12): 2422-2435.
Holt, R.D. 1996. Evidence for a Late Cainozoic Flood/post-Flood boundary. Journal of Creation 10(1):128-167.
Ivany, L.C., and B. Runnegar. 2010. Early Permian seasonality from bivalve d18O and implications for the oxygen isotopic composition of seawater. Geology 38(11): 1027-1030.
Jones, D.S. 1981. Repeating layers in the molluscan shell are not always periodic. Journal of Paleontology 55: 1076-1082.
Jones, D.S., M.A. Arthur, and D.J. Allard. 1989. Sclerochronological records of temperature and growth from shells of Mercenaria mercenaria from Narragansett Bay, Rhode Island. Marine Biology 102(2) :225-234.
Jones, D.S., and I.R. Quitmyer. 1996. Marking time with bivalve shells: oxygen isotopes and season of annual increment formation. Palaios 11: 340-346.
Killam, D. 2018. The when, how and why of Bivalve shell growth: sclerochronology as a tool to understand physiology in Jurassic and future oceans. Ph.D. dissertation, University of California Santa Cruz, Santa Cruz, CA.
Kirby, M.X. 2000. Paleoecological differences between Tertiary and Quaternary Crassostrea oysters, as revealed by stable isotope sclerochronology. Palaios 15(2): 132-141.
Kirby, M.X. 2001. Differences in growth rate and environment between Tertiary and Quaternary Crassostrea oysters. Paleobiology 27(1): 84-103.
Kirby, M.X., and J.B.C. Jackson. 2004. Extinction of a fast-growing oyster and changing ocean circulation in Pliocene tropical America. Geology 32(12): 1025-1028.
Kusnerick, K.M., R. Lockwood, and A.N. Grant. 2018. Using the Fossil Record to Establish a Baseline and Recommendations for Oyster Mitigation in the Mid-Atlantic U.S. In Marine Conservation Paleobiology, Topics in Geobiology 47.
Lawrence, D.R. 1991. The neotype of Crassostrea gigantissima (Finch, 1824). Journal of Paleontology 65(2): 342-343.
Lee, W.-S., P. Monaghan, and N.B. Metcalfe. 2013. Experimental demonstration of the growth rate-lifespan trade-off. Proceedings of the Royal Society B 280(1752): 1-8.
Lindstedt, S. 1981. Body size, physiological time, and longevity of homeothermic animals. The Quarterly Review of Biology 56(1): 1-16.
López, R.E. 1998. The antediluvian patriarchs and the Sumerian King List. Journal of Creation 12(3): 347-357.
Marchionni, S., C. Sell, and A. Lorenzini. 2020. Development and longevity: Cellular and molecular determinants - a mini-review. Gerontology 66(3): 223-230.
Miller, R.A., J.M. Harper, R.C. Dysko, S.J. Durkee, and S.N. Austad. 2002. Longer life spans and delayed maturation in wild-derived mice. Experimental Biological Medicine (Maywood) 227(7): 500-508.
Morris, H.M. 1993. The Genesis Record: A Scientific and Devotional Commentary on the Book of Beginnings (23 rd printing). Baker Book House, Grand Rapids, MI
Morris, J.D., and F.J. Sherwin. 2011. The Fossil Record (3 rd printing). Institute for Creation Research, Dallas, TX.
Moss, D.K., L.C. Ivany, and D.S. Jones. 2021. Fossil bivalves and the sclerochronological reawakening. Paleobiology 47(4): 551-573.
Moss, D.K., L.C. Ivany, E.J. Judd, P.W. Cummings, C.E. Bearden, W.-J. Kim, E.G. Artruc, and J.R. Driscoll. 2016. Lifespan, growth rate, and body size across latitude in marine Bivalvia, with implications for Phanerozoic evolution. Proceedings of the Royal Society B 283, 20161364: 1-7.
Moss, D. K., L.C. Ivany, R.B. Silver, J. Schue, and E.G. Artruc. 2017. High-latitude settings promote extreme longevity in fossil marine bivalves. Paleobiology 43(3): 365-382.
Mueller, U., and A. Mazur. 2009. Tallness comes with higher mortality in two cohorts of US army officers. In Proceedings of the Population Association of America Meeting (annual meeting). PPA, Detroit, MI.
Nehring, S. 2011. NOBANIS - Invasive Alien Species Fact Sheet - Crassostrea gigas . https://www.nobanis.org/globalassets/speciesinfo/c/crassostrea-gigas/crassostrea-gigas.pdf (posted on nobanis.org; accessed October 16, 2023).
Nelson, V. 2017. Monumental Monsters. Untold Secrets of Planet Earth Publishing Company, Inc., Red Deer, Alberta, Canada.
Norton, C.C. 2021. Size and age structure of modern Crassostrea virginica in lower Chesapeake Bay. Geology Senior Theses, Paper 12. William & Mary, Williamsburg, VA.
Oard, M.J. 1984. Ice Ages: The Mystery Solved?-Part II: The Manipulation of Deep-Sea Cores. Creation Research Society Quarterly 21(3): 125-137.
Oard, M.J. 1990. An Ice Age Caused by the Genesis Flood. Institute for Creation Research, El Cajon, CA.
Oard, M.J. 2002a. Dealing carefully with the data. Journal of Creation 16(1): 68-72.
Oard, M.J. 2002b. Wild ice-core interpretation by uniformitarian scientists. Journal of Creation 16(1): 45-47.
Oard, M.J. 2003. The 'cool-tropics paradox' in palaeoclimatology. Journal of Creation 17(1): 6-8.
Oard, M.J. 2005. The Frozen Record. Institute for Creation Research, Santee, CA.
Oard, M.J. 2013. Geology indicates the terrestrial Flood/post-Flood boundary is mostly in the Late Cenozoic. Journal of Creation 27(1): 119-127.
Osborne, P. 1999. Crassostrea virginica. Animal Diversity Web. University of Michigan Museum of Zoology. https://animaldiversity.org/accounts/Crassostrea_virginica/ (accessed August 30, 2023).
Overman, R. 2021. Letters to the Editor: Biblical Science. Creation Research Society Quarterly 57(3): 236-238.
Palmer, K.L., D.K. Moss, D. Surge, and S. Turek. 2021. Life history patterns of modern and fossil Mercenaria spp. from warm vs. cold climates. Palaeogeography, Palaeoclimatology, Palaeoecology 566, 110227: 1-13.
Patten, D.W. 1982. The longevity accounts in ancient history. Creation Research Society Quarterly 19(1): 40-52.
Powell, E.N., J.M. Klinck, X. Guo, S.E. Ford, and D. Bushek. 2011. The potential for oysters, Crassostrea vriginica, to develop resistance to dermo disease in the field: Evaluation using a gene-based population dynamics model. Journal of Shellfish Research 30(3): 685-712.
Reichard, M., and M. Polacik. 2019. The natural history of model organisms: Northobranchius furzeri, an 'instant' fish from an ephemeral habitat. eLife 8: e41548.
Reid, K., M.A. Bell, and K.R. Veeramah. 2021. Threespine Stickleback: A model system for evolutionary genomics. Annual Review of Genomics and Human Genetics 22: 357-3836.
Ricklefs, R.E. 2010a. Life-history connections to rates of aging in terrestrial vertebrates. PNAS 107(22): 10,314-10,319.
Ricklefs, R.E. 2010b. Embryo growth rates in birds and mammals. Functional Ecology 24(3): 588-596.
Ridgway, I.D., C.A. Richardson, and S.N. Austad. 2011. Maximum shell size, growth rate, and maturation age correlate with longevity in bivalve molluscs. The Journals of Gerontology: Series A 66A(2): 183-190.
Samaras, T.T. 2014. Evidence from eight different types of studies showing that smaller body size is related to greater longevity . Journal of Scientific Research and Reports 3(16): 2150-2160.
Sato, S. 1994. Analysis of the relationship between growth and sexual maturation in Phacosoma japonicum (Bivalvia: Veneridae). Marine Biology 118: 663-672.
Schmidt-Nielsen, K. 1986. Scaling: Why Is Animal Size So Important? (3 rd printing). Cambridge University Press, Cambridge, England.
Sohl, N.F., and E.G. Kauffman. 1964. Giant upper Cretaceous oysters from the Gulf Coast and Caribbean. Geological Survey Professional Paper 483-H . United States Government Printing Office, Washington, D.C.
Spencer, R.S., and L.D. Campbell. 1987. The fauna and paleoecology of the late Pleistocene marine sediments of southeastern Virginia. Bulletins of American Paleontology 92: 1-124. Cited in Kirby (2001).
Tabatabaie, V., G. Atzmon, S.N. Rajpathak, R. Freeman, N. Barzilai, and J. Crandall. 2011. Exceptional longevity is associated with decreased reproduction. Aging (Albany NY) 3(12): 1202-1205.
Trofimova, T., S.J. Alexandroff, M.J. Mette, et al. 2020. Fundamental questions and applications of sclerochronology: Community-defined research priorities. Estuarine, Coastal and Shelf Science 245(106977).
Vardiman, L. 2001. Climates Before and After the Genesis Flood: Numerical Models and Their Implications . Institute for Creation Research El Cajon, CA.
von Bertalanffy, L. 1938. A quantitative theory of organic growth (inquiries on growth laws II). Human Biology 10: 181-213.
Wallace, R.K. 2022. The life cycle of the Eastern Oyster. Barnegat Bay Shellfish . Auburn University Marine Extension and Research Center, Mobile, AL. https://barnegatshellfish.org/oyster_lifecycle_02.htm (accessed August 30, 2023).
Wasser, D.E., and P.W. Sherman. 2010. Avian longevities and their interpretation under evolutionary theories of senescence. Journal of Zoology 280(2): 103-155.
West, G.B., J.H. Brown, and B.J. Enquist. 2001. A general model for ontogenetic growth. Nature 413(6856): 628-631.
Whitcomb, J.C., and H.M. Morris. 1991. The Genesis Flood: The Biblical Record and Its Scientific Implications . 35 th printing. Presbyterian and Reformed Publishing Company, Phillipsburg, New Jersey.
Woodmorappe, J. 1996. Noah's Ark: A Feasability Study. Institute for Creation Research Santee, CA.
Woodmorappe, J. 2003. Collapsing the long bristlecone pine tree ring chronologies. In R.L. Ivey, Jr. (editor). Proceedings of the 5 th International Conference on Creationism , pp. 491-504. Creation Science Fellowship, Pittsburgh, PA.
Woodmorappe, J. 2009. Biblical chronology and the 8,000-year-long bristlecone pine tree-ring chronology. Answers in Depth 4: 4-5.
Woodmorappe, J. 2018. Tree ring disturbance clustering for the collapse of long tree-ring chronologies. In J. H. Whitmore (editor). Proceedings of the 8 th International Conference on Creationism , pp. 652-672. Creation Science Fellowship Pittsburgh, PA.
Wormser, D.E. Di Angelantonio, S. Kaptoge, et al. 2012. Adult height and risk of cause-specific death and vascular morbidity in 1 million people: individual participant meta-analysis. International Journal of Epidemiology 41(5): 1419-1433.
Zhang, Y.G., M. Pagani, and Z. Liu. 2014. A 12-million-year temperature history of the tropical Pacific Ocean. Science 344(6179): 84-87.
Zimmt, J.B., R. Lockwood, C.F.T. Andrus, and G.S. Herbert. 2019. Sclerochronological basis for growth band counting: A reliable technique for life-span determination of Crassostrea virginica from the mid-Atlantic United States. Palaeogeography, Palaeoclimatology, and Palaeoecology 516: 54-63.
Tables
Table I. Approximate maximum observed body masses and lifespans, as well as ages at first and full sexual maturity, for six Modern assemblages of the Japanese venerid bivalve Phacosoma japonicum (since reclassified as Dosinia japonica) compiled from data and figures in Sato (1994).
Locality |
Max. Observed Mass (g) |
1 st tsex (years) |
Full t sex(years) |
Max. Lifespan (years) |
Ishikari Bay |
63 |
>5 |
7 |
12 |
Hakodate Bay |
53 |
4 |
5 |
11 |
Ariake Bay |
45 |
4 |
5 |
9 |
Tokyo Bay |
27 |
3 |
4 |
8 |
Seto Inland Sea |
21 |
3 |
4 |
7 |
Kagoshima |
16 |
2 |
3 |
7 |
Table II. Caption. Results of Mann-Whitney U tests for differences in shell heights between Late Pleistocene (LP), Middle Pleistocene (MP), colonial, and modern Crassostrea specimens. Statistically significant differences are highlighted in bold. After Table II from Kusnerik et al. (2018).
Maryland |
Virginia |
North Carolina |
|
MP vs. LP |
- |
- |
Z225,21= -2.93 p = 0.003 |
MP vs. Colonial |
- |
Z647,364= -4.48 p < 0.0001 |
- |
MP vs. Modern |
- |
Z647,6916= -15.15 p < 0.0001 |
Z21,5443= -1.05 p < 0.29 |
LP vs. Colonial |
Z36,3= -1.69 p = 0.09 |
- |
- |
LP vs. Modern |
Z36,1176= -6.44 p < 0.0001 |
- |
Z225,5443= -3.76 p < 0.0001 |
Colonial vs. Modern |
Z 3,1176 = -0.69 p = 0.49 |
Z364,6916= -9.56 p < 0.0001 |
Table III. Uniformitarian age assignments and locations from which Crassostrea specimens were collected from the tropical Americas. The C. aff. C. virginica specimens are of uncertain taxonomic classification.
Conventional Age Assignment |
Species |
Location(s) |
Recent |
C. virginica |
Venezuela and Trinidad |
Recent |
C. columbiensis |
Panama |
Holocene |
C. virginica |
Panama |
Late Pleistocene |
C. virginica |
Panama |
Pleistocene |
C. virginica |
Costa Rica, Venezuela, Barbados, Panama |
Pleistocene |
C. columbiensis |
Panama |
Early Pleistocene |
C. virginica |
Trinidad |
Pliocene |
C. aff. C. virginica |
Trinidad, Venezuela, and Jamaica |
Pliocene |
C. cahobasensis |
Venezuela |
Early Pliocene |
C. cahobasensis |
Venezuela |
Late Miocene |
C. cahobasensis |
Panama and Venezuela |
Miocene |
C. cahobasensis |
Panama |
Figure Captions
Figure 1. The oyster species Crassostrea virginica, or Eastern Oyster. Figure 1a by Andrew C, Creative Commons Attribution 2.0 Generic license. Figure 1b by Eric A. Lazo-Wasem, , Yale University Peabody Museum. Creative Commons CC0 1.0 Universal Public Domain Dedication.
Figure 2. The von Bertalanffy growth curve, showing the increase of an organism's length or height as a function of time since birth or hatching. Note that growth effectively, if not completely, stops, when the organism reaches adulthood at time tmature.
Figure 3. Longevity studies have shown that greater longevity is associated with larger adult body sizes and longer maturation times.
Figure 4. Average shell heights for Late Pleistocene, colonial, and modern Crassostrea virginica specimens from Maryland. Error bars represent the standard error. Image credit: Richard Overman. After Figure 6a in Kusnerik et al. (2018).
Figure 5. Average shell heights for Middle Pleistocene, colonial, and modern Crassostrea virginica specimens from Virginia. Error bars represent the standard error. Image credit: Richard Overman. After Figure 7a in Kusnerik et al. (2018).
Figure 6. Average shell heights for Middle Pleistocene, Late Pleistocene, and modern Crassostrea virginica specimens from North Carolina. Error bars represent the standard error. Image credit: Richard Overman. After Figure 8a in Kusnerik et al. (2018).
Figure 7. Maximum lifespans (large black dots) for four assemblages of California, North Carolina, and Virginia Crassostreaoysters, all collected between 34.6 and 36.8° north latitude. Maximum lifespans for the two Miocene (Crassostrea titan) fossil assemblages are much greater than those for the Late Pleistocene and 'Recent' (Crassostrea virginica) assemblages. Small black dots represent the average age of each assemblage, and error bar half-widths are standard deviations in assemblage ages. The number of fossil specimens in each assemblage is indicated.
Figure 8. Height-versus-age growth curves constructed for the four Crassostrea oyster assemblages whose age data are summarized in Figure 7. After Figure 3B in Kirby (2001).
Figure 9. Valve height statistical data for the four Crassostrea oyster assemblages whose growth curves are shown in Figure 8. Dark black dots indicate maximum heights, small black dots indicate averages, and error bar half-widths represent height standard deviations. The number of specimens in each assemblage is indicated.
Figure 10. Crassostrea gigantissima fossil. Image Credit: Wilson44691. Creative Commons BY-SA 3.0 License.
Figure 11. Figure 10 in Harzhauser et al. (2016), depicting the growth curve for Crassostrea gryphoides (now Magallana gryphoides ) constructed from 1121 Early Miocene shells (Austria). Creative Commons Attribution 3.0 License. Growth curves labelled 1 are the same Miocene growth curves shown in Figure 8. Growth curve 2 is for C. madrasensis (recent, Bangladesh), growth curve 4 is for C. corteziensis (recent, Mexico), and growth curve 5 is for Crassostrea gigas, or Magallana gigas (recent, France). Two of the curves labelled "3" are the two C. virginica curves from Kirby (2001) shown in Figure 8, with the third such curve from a Chesapeake and Delaware Bay study of recent C. virginica by Powell et al. (2011). Despite the broad geographical distribution of the Recent oysters, their growth curves are all consistent with accelerated maturation and smaller body sizes compared to the Miocene specimens (curves 1).
Figure 12. Figure 3 from Kirby and Jackson (2004), showing that juvenile Pliocene and Miocene C. cahobasensisspecimens have larger body sizes at all stages of ontogenetic development compared to Recent and Pleistocene C. columbiensis and C. virginica specimens, regardless of whether estimated carbonate shell content (a) or total estimated biomass (b) is used as a measurement of size. Error bars represent 95% confidence intervals. Fair Use Application, used by general permission of the Geological Society of America.